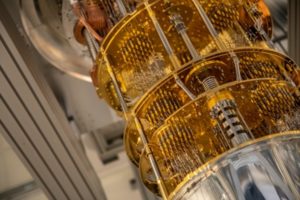
DOE’s Lawrence Berkeley National Laboratory is using a sophisticated cooling system to keep qubits – the heart of quantum computers – cold enough for scientists to study them for future use in quantum computers.
To use quantum computers on a large scale, we need to improve the technology at their heart – qubits. Qubits are the quantum version of conventional computers’ most basic form of information, bits. The DOE’s Office of Science is supporting research into developing the ingredients and recipes to build these challenging qubits.
At the atomic scale, physics gets very weird. Electrons, atoms, and other quantum particles interact with each other differently than ordinary objects. In certain materials, we can harness these strange behaviors. Several of these properties – particularly superposition and entanglement – can be extremely useful in computing technology.
The principle of superposition is the idea that a qubit can be in multiple states at once. With traditional bits, you only have two options: 1 or 0. These binary numbers describe all of the information in any computer. Qubits are more complicated.
Imagine a pot with water in it. When you have water in a pot with a top on it, you don’t know if it’s boiling or not. Real water is either boiling or not; looking at it doesn’t change its state. But if the pot was in the quantum realm, the water (representing a quantum particle) could both be boiling and not boiling at the same time or any linear superposition of these two states. If one took the lid off of that quantum pot, the water would immediately be one state or the other. The measurement forces the quantum particle (or water) into a specific observable state.
Entanglement is when qubits have a relationship to each other that prevents them from acting independently. It happens when a quantum particle has a state (such as spin or electric charge) that’s linked to another quantum particle’s state. This relationship persists even when the particles are physically far apart, even far beyond atomic distances.
These properties allow quantum computers to process more information than conventional bits that can only be in a single state and only act independently from each other.
Harnessing Quantum Properties
But to get any of these great properties, you need to have fine control over a material’s electrons or other quantum particles. In some ways, this isn’t so different from conventional computers. Whether electrons move or not through a conventional transistor determines the bit’s value, making it either 1 or 0.
Rather than simply switching electron flow on or off, qubits require control over tricky things like spin. To create a qubit, scientists have to find a spot in a material where they can access and control these quantum properties. Once they access them, they can then use light or magnetic fields to create superposition, entanglement, and other properties.
In many materials, scientists do this by manipulating the spin of individual electrons. Electron spin is similar to the spin of a top; it has a direction, angle, and momentum. Each electron’s spin is either up or down. But as a quantum mechanical property, spin can also exist in a combination of up and down. To influence electron spin, scientists apply microwaves (similar to the ones in your microwave oven) and magnets. The magnets and microwaves together allow scientists to control the qubit.
Since the 1990s, scientists have been able to gain better and better control over electron spin. That’s allowed them to access quantum states and manipulate quantum information more than ever before.
To see where that’s gone today, it’s remarkable,” said David Awschalom, a quantum physicist at DOE’s Argonne National Laboratory and the University of Chicago as well as Director of the Chicago Quantum Exchange.
Whether they use electron spin or another approach, all qubits face major challenges before we can scale them up. Two of the biggest ones are coherence time and error correction.
When you run a computer, you need to be able to create and store a piece of information, leave it alone, and then come back later to retrieve it. However, if the system that holds the information changes on its own, it’s useless for computing. Unfortunately, qubits are sensitive to the environment around them and don’t maintain their state for very long.
Right now, quantum systems are subject to a lot of “noise,” things that cause them to have a low coherence time (the time they can maintain their condition) or produce errors. “Making sure that you get the right answer all of the time is one of the biggest hurdles in quantum computing,” said Danna Freedman, an associate professor in chemistry at Northwestern University.
Even if you can reduce that noise, there will still be errors. “We will have to build technology that is able to do error correction before we are able to make a big difference with quantum computing,” said Giulia Galli, a quantum chemist and physicist at DOE’s Argonne National Laboratory and the University of Chicago.
The more qubits you have in play, the more these problems multiply. While today’s most powerful quantum computers have about 50 qubits, it’s likely that they will need hundreds or thousands to solve the problems that we want them to.
Exploring Options
The jury is still out on which qubit technology will be the best. “No real winner has been identified,” said Galli. “[Different ones] may have their place for different applications.” In addition to computing, different quantum materials may be useful for quantum sensing or networked quantum communications.
To help move qubits forward, DOE’s Office of Science is supporting research on a number of different technologies. “To realize quantum computing’s enormous scientific potential, we need to reimagine quantum R&D by simultaneously exploring a range of possible solutions,” said Irfan Siddiqi, a quantum physicist at the DOE Lawrence Berkeley National Laboratory and the University of California, Berkeley.
Superconducting Qubits
Superconducting qubits are currently the most advanced qubit technology. Most existing quantum computers use superconducting qubits, including the one that “beat” the world’s fastest supercomputer. They use metal-insulator-metal sandwiches called Josephson junctions. To turn these materials into superconductors – materials that electricity can run through with no loss – scientists lower them to extremely cold temperatures. Among other things, pairs of electrons coherently move through the material as if they’re single particles. This movement makes the quantum states more long-lived than in conventional materials.
To scale up superconducting qubits, Siddiqi and his colleagues are studying how to build them even better with support from the Office of Science. His team has examined how to make improvements to a Josephson junction, a thin insulating barrier between two superconductors in the qubit. By affecting how electrons flow, this barrier makes it possible to control electrons’ energy levels. Making this junction as consistent and small as possible can increase the qubit’s coherence time. In one paper on these junctions, Siddiqi’s team provides a recipe to build an eight-qubit quantum processor, complete with experimental ingredients and step-by-step instructions.
Qubits Using Defects
Defects are spaces where atoms are missing or misplaced in a material’s structure. These spaces change how electrons move in the materials. In certain quantum materials, these spaces trap electrons, allowing researchers to access and control their spins. Unlike superconductors, these qubits don’t always need to be at ultra-low temperatures. They have the potential to have long coherence times and be manufactured at scale.
While diamonds are usually valued for their lack of imperfections, their defects are actually quite useful for qubits. Adding a nitrogen atom to a place where there would normally be a carbon atom in diamonds creates what’s called a nitrogen-vacancy center. Researchers using the Center for Functional Nanomaterials, a DOE Office of Science user facility, found a way to create a stencil just two nanometers long to create these defect patterns. This spacing helped increase these qubits’ coherence time and made it easier to entangle them.
But useful defects aren’t limited to diamonds. Diamonds are expensive, small, and hard to control. Aluminum nitride and silicon carbide are cheaper, easier to use, and already common in everyday electronics. Galli and her team used theory to predict how to physically strain aluminum nitride in just the right way to create electron states for qubits. As nitrogen vacancies occur naturally in aluminum nitride, scientists should be able to control electron spin in it just as they do in diamonds. Another option, silicon carbide, is already used in LED lights, high-powered electronics, and electronic displays. Awschalom’s team found that certain defects in silicon carbide have coherence times comparable to or longer than those in nitrogen-vacancy centers in diamonds. In complementary work, Galli’s group developed theoretical models explaining the longer coherence times.
Based on theoretical work, we began to examine these materials at the atomic scale. We found that the quantum states were always there, but no one had looked for them,” said Awschalom. “Their presence and robust behavior in these materials were unexpected. We imagined that their quantum properties would be short-lived due to interactions with nearby nuclear spins.” Since then, his team has embedded these qubits in commercial electronic wafers and found that they do surprisingly well. This can allow them to connect the qubits with electronics.
Materials by Design
While some scientists are investigating how to use existing materials, others are taking a different tack – designing materials from scratch. This approach builds custom materials molecule by molecule. By customizing metals, the molecules or ions bound to metals, and the surrounding environment, scientists can potentially control quantum states at the level of a single particle.
When you’re talking about both understanding and optimizing the properties of a qubit, knowing that every atom in a quantum system is exactly where you want it is very important,” said Freedman.
With this approach, scientists can limit the amount of nuclear spin (the spin of the nucleus of an atom) in the qubit’s environment. A lot of atoms that contain nuclear spin cause magnetic noise that makes it hard to maintain and control electron spin. That reduces the qubit’s coherence time. Freedman and her team developed an environment that had very little nuclear spin. By testing different combinations of solvents, temperatures, and ions/molecules attached to the metal, they achieved a 1 millisecond coherence time in a molecule that contains the metal vanadium. That was a much longer coherence time than anyone had achieved in a molecule before. While previous molecular qubits had coherence times that were five times shorter than diamond nitrogen-vacancy centers’ times, this matched coherence times in diamonds.
“That was genuinely shocking to me because I thought molecules would necessarily be the underdogs in this game,” said Freedman. “[It] opens up a gigantic space for us to play in.”
The surprises in quantum just keep coming. Awschalom compared our present-day situation to the 1950s when scientists were exploring the potential of transistors. At the time, transistors were less than half an inch long. Now laptops have billions of them. Quantum computing stands in a similar place.
The overall idea that we could completely transform the way that computation is done and the way nature is studied by doing quantum simulation is really very exciting,” said Galli. “Our fundamental way of looking at materials, based on quantum simulations, can finally be useful to develop technologically relevant devices and materials.”
Source: DOE